Graphene – a single layer of ordinary graphite – is just one atom thick, and yet it is one of the strongest materials ever measured. Since its discovery by Andre Geim and Konstantin Novoselov (earning them the 2010 Nobel Prize in Physics) (1), graphene has been the centre of a highly active field in materials research (2,3). Remarkably, while still being studied fundamentally by theorists and experimentalists in many fields, graphene has already made its way into several applications (4,5).
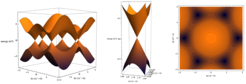
Figure 1: Calculated band structure of graphene utilizing a tight-binding approach. The and bands only touch at the K points at the corners of the Brillouin zone. At these points, the characteristic Dirac cones form. This model considers nearest neighbor hopping (2.8 eV) with a lattice constant of 2.46.
Our research focuses on the study of graphene’s fundamental properties, as well as how to manipulate these in situ. The charge carrier density, for example, can be controlled by depositing alkali metal atoms on the graphene surface, which also modifies the interaction between electrons and vibrations of the graphene lattice (6). This is the same interaction responsible for conventional superconductivity, and in 2015, we witnessed signatures of superconductivity in lithium-covered graphene in an ARPES experiment (7). We also study the effects of depositing heavier atoms, such as thallium, which affects the scattering behaviour of the electrons (8); and gadolinium, which gives access to a singularity in the density of states near the Fermi level, possibly leading to novel exotic physics in graphene (9). In the very dilute regime of adatoms, we are interested in the recently experimentally confirmed Kekulé order (10) and its connection to superconductivity and charge density type orders.
References:
1. The Nobel Prize in Physics 2010 [Internet]. [cited 2015 Mar 3]. Available from: http://www.nobelprize.org/nobel_prizes/physics/laureates/2010/
2. Novoselov KS, Jiang D, Schedin F, Booth TJ, Khotkevich VV, Morozov SV, et al. Two-dimensional atomic crystals. Proc Natl Acad Sci U S A. 2005 Jul 26;102(30):10451–3.
3. Geim AK. Graphene: Status and Prospects. Science. 2009 Jun 19;324(5934):1530–4.
4. Stankovich S, Dikin DA, Dommett GHB, Kohlhaas KM, Zimney EJ, Stach EA, et al. Graphene-based composite materials. Nature. 2006 Jul 20;442(7100):282–6.
5. Bonaccorso F, Sun Z, Hasan T, Ferrari AC. Graphene photonics and optoelectronics. Nat Photonics. 2010 Sep;4(9):611–22.
6. Profeta G, Calandra M, Mauri F. Phonon-mediated superconductivity in graphene by lithium deposition. Nat Phys. 2012 Feb;8(2):131–4.
7. Ludbrook BM, Levy G, Zonno M, Schneider M, Dvorak DJ, Veenstra CN, et al. Evidence for superconductivity in Li-decorated graphene. submitted. 2015;
8. Straßer C, Ludbrook BM, Levy G, Macdonald AJ, Burke SA, Wehling TO, et al. Long- versus Short-Range Scattering in Doped Epitaxial Graphene. Nano Lett. 2015 Mai;15(5):2825–9.
9. Nandkishore R, Levitov LS, Chubukov AV. Chiral superconductivity from repulsive interactions in doped graphene. Nat Phys. 2012 Feb;8(2):158–63.
10. Gutiérrez C, Kim C-J, Brown L, Schiros T, Nordlund D, Lochocki EB, et al. Imaging chiral symmetry breaking from Kekule bond order in graphene. Nat Phys. 2016 Oct;12(10):950–8.
Principal investigators / Participating scientists:
Andrea Damascelli damascelli@physics.ubc.ca
Ulrich Starke U.Starke@fkf.mpg.de
Sarah Burke saburke@phas.ubc.ca
Joshua Folk jfolk@phas.ubc.ca
Christian Ast c.ast@fkf.mpg.de